Lecture 1 Overview of Observational Cosmology
This section introduces the observational foundation of Cosmology. It builds upon the material in PH30111: Introduction to Galaxies and Cosmology. Some material may be familiar to you, but the origins and derivations of the equations will be discussed in more depth, and will include the relativistic treatment introduced in the first half of this course.
1.1 The Cosmological Principle
The Cosmological Principle is the belief that our position in the Universe is not special in any way.
If the Cosmological Principle holds, then there is no special position in the Universe, meaning that it should be the same everywhere (i.e. it should be homogeneous), and it should have no preferred direction (i.e. it should be isotropic)
Clearly the Universe is not isotropic and homogeneous on small scales – the distributions of things like stars, planets and galaxies give more or less dense regions locally. But on a large scale, the Universe does display homogeneity and isotropy; Galaxy clusters are spread over the Universe and not concentrated to one side, and the CMB has only very tiny fluctuations.
1.2 A brief history of the Universe
The ‘Cosmic Calendar’ shown in Figure 1.1 condenses the evolution of the Universe to a time-scale of 1 calendar year. If the Big Bang occurred on January 1st, human life wouldn’t exist until 11:52pm on 31st December! The formation of the Solar System would have occurred in September, and our home in the Galaxy, the disk of the Milky Way, would have formed in May.
As Figure 1.1 shows, our understanding of how the Universe and our place in it has evolved over time uses different sources of evidence depending on the time frame. Our knowledge of recent history comes from written records, while our knowledge of the formation of the Solar System and the evolution of Earth comes from geology. However, to understand how the Universe as a whole came to be, we must look further back in time.
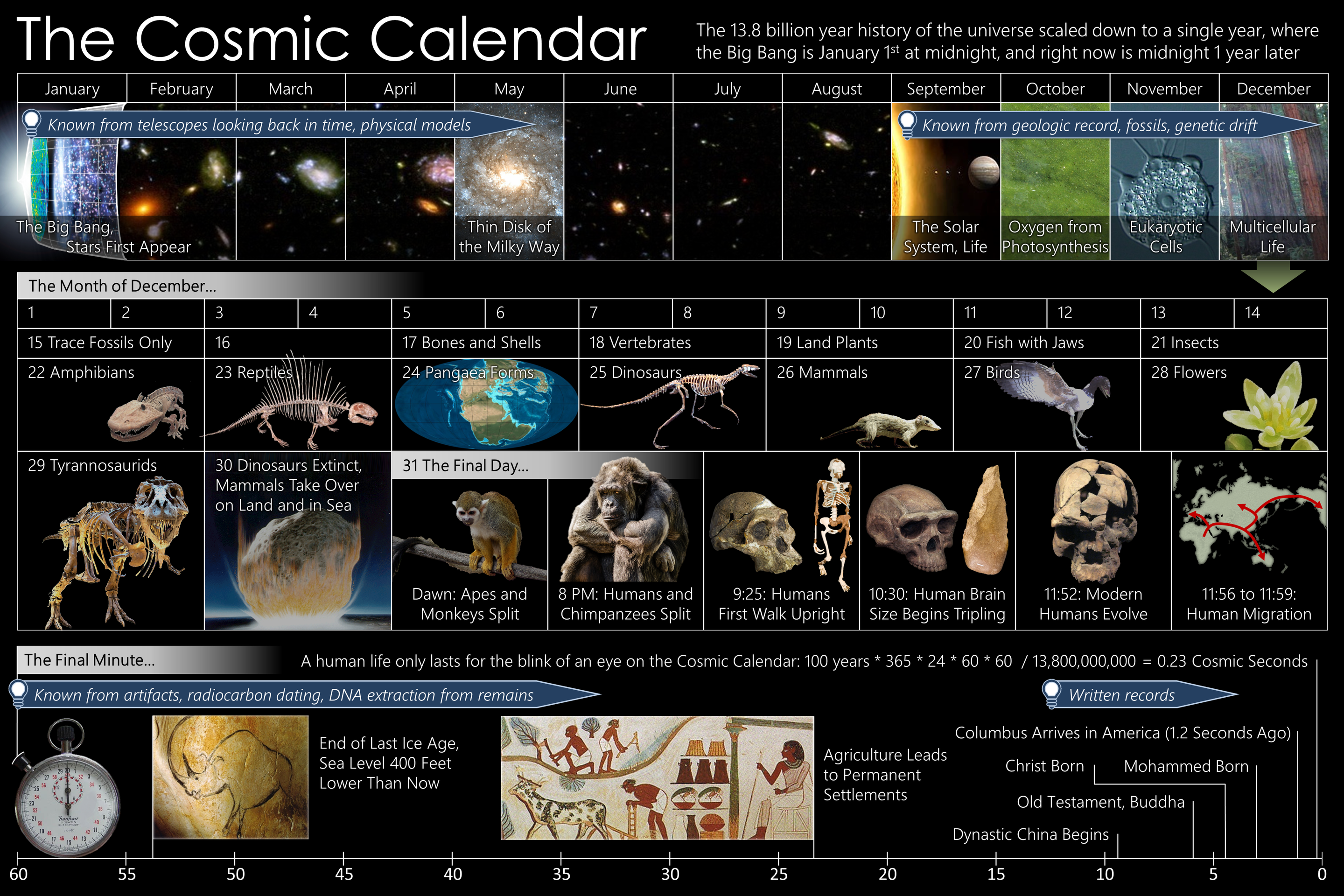
Figure 1.1: The Cosmic Calendar. Credit: By Efbrazil, CC BY-SA 3.0.
Figure 1.2 illustrates our current understanding of the evolution of the Universe, starting with the Big Bang, through to the Universe as we see it today. This section gives a brief history of the evolution; topics will be covered in further detail later in the course. We will not move through these chronologically in this course; instead this is intended to give you an overview of how the different ideas we cover come together.
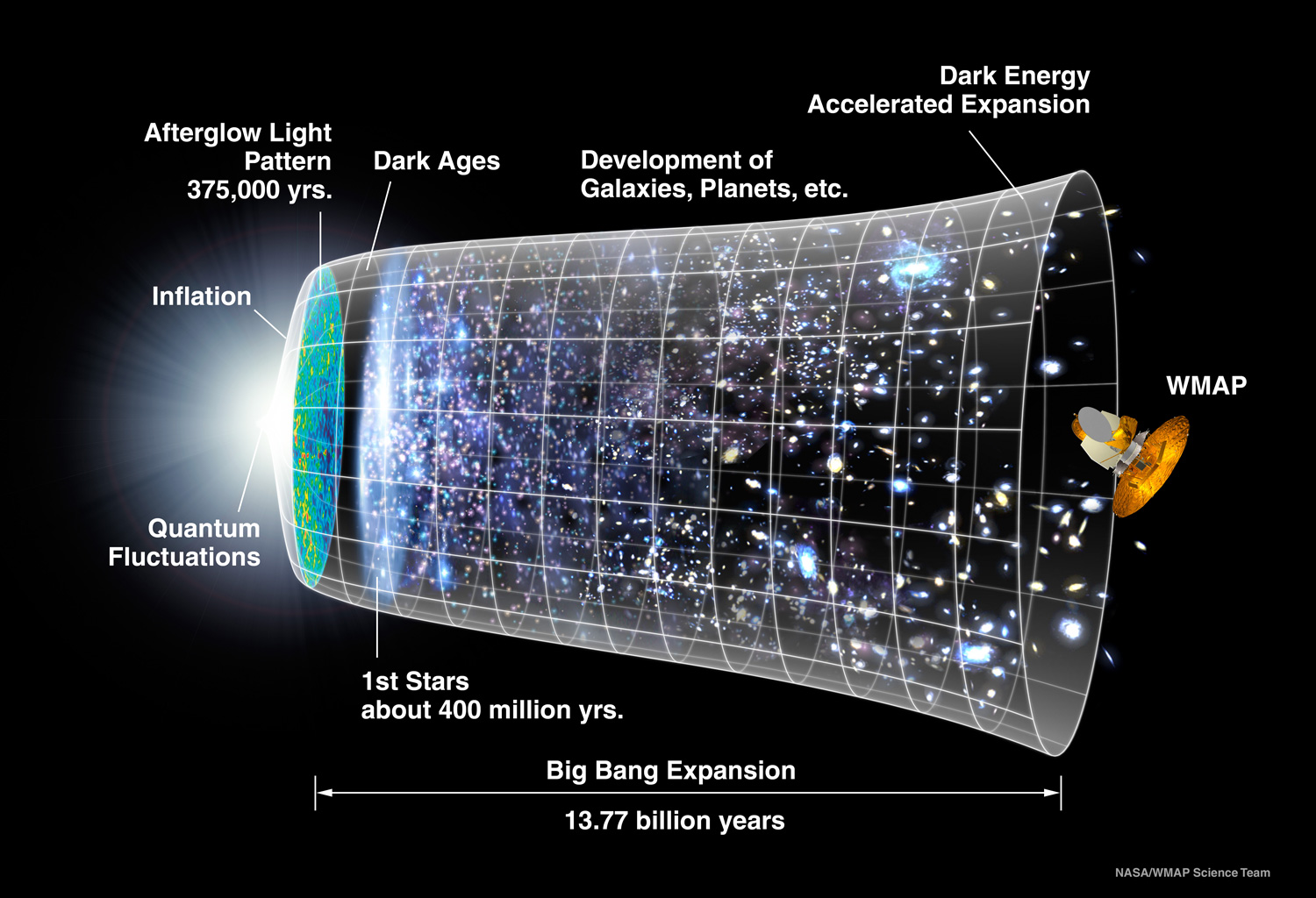
Figure 1.2: Evolution of the Universe. Original image credit: NASA/WMAP Science Team
1.3 The first second
The first second after the Big Bang was quite dramatic. Highlights of this time are given below. Bear in mind that all of the following happens in less time than it takes for your heart to beat twice.
- The Big Bang \((t = 0)\)
The Universe began with the Big Bang, rapidly expanding from an initial singularity.
- Planck epoch \((t = 10^{-43}~s)\)
The Planck epoch refers to the stage when the Universe’s density exceeded a critical density, such that the energy scale is greater than the Planck scale. At this scale, quantum effects are important even for gravitational physics.
- Separation of forces \((10^{-43}~s < t < 10^{-36}~s)\)
After the Planck epoch, other fundamental forces start to separate out. First, the gravitational force and electronuclear force decouple, then the electronuclear force separates out into the strong and electroweak forces.
- Inflation \((t < 10^{-32}~s)\)
Early in its evolution, the Universe underwent a period of rapid inflation, expanding by a factor of \(10^{78}\) (or \(10^{26}\) in each of the three spatial dimensions). Under this rapid expansion, an area of space of the order of 1 nm\(^{3}\) would expand to approximately 30 pc\(^{3}\) (equivalent to around 100 lyr\(^{3})\). Such expansion must occur at greater than the speed of light (\(c\)). However, as it is the metric of space time that is expanding, the limitation of exceeding \(c\) is not applicable, and inflation can occur.
- Electroweak symmetry breaking \((t = 10^{-12}~s)\)
After inflation, the electroweak force separates into the electromagnetic and weak nuclear forces.
- Quark epoch \((t = 10^{-12}~s)\)
At the same time as electroweak symmetry breaking, the energy density of the Universe has decreased such that matter can exist in the form of quarks.
- Baryogenesis \((t = 10^{-11}~s)\)
Baryogenesis is the stage where matter can start to exist in the form of baryons - e.g. protons, neutrons. Baryogenesis should have produced both baryons and anti-baryons (e.g. protons and anti-protons). Current evidence - i.e. the fact that we exist and are composed of baryons - shows that there must have been an asymmetry in the proportions of baryons/anti-baryons, otherwise they would have all have annihilated. The Baryogenesis process and baryon asymmetry are current areas of research.
- Hadron epoch \((10^{-6}~s < t < 1~s)\)
The Hadron epoch occurred when the Universe had cooled far enough for hadrons to exist and dominate the energy density. The proportions of hadrons and anti-hadrons were similar, such that they were in thermal equilibrium and mostly annihilated, producing photons, although a small number of hadrons remained.
- Neutrino decoupling \((t < 1~s)\)
Around 1s after the Big Bang, neutrinos decoupled and were free to propagate through the Universe. Neutrinos have a very small interaction cross section, so the Cosmic Neutrino Background created at neutrino decoupling should still exist today. However, as the neutrinos produced at decoupling have such low energies, it is impossible to detect them with current experiments.
- Lepton epoch \((1~s < t < 10~s)\)
Similar to the Hadron epoch, the Lepton epoch produced both leptons and anti-leptons (e.g, electrons, muons, neutrinos etc.) These would have annihilated, producing pairs of photons. A small number of leptons remain after annihilation.
1.4 After the first second:
The Universe has now been evolving for around 1 s. It has exponentially expanded, created all the matter and radiation we see (and destroyed a whole load too), and gone through several rounds of fundamental physical forces. What else is left to do for the next 13.77 billion years?
- Photon epoch \((10~s < t < 377,000\text{ years})\)
After baryons, hadrons and leptons have mostly been annihilated, the majority of the Universe’s energy is dominated by photons.
- Light Element Nucleosynthesis \((2 \text{ mins} < t < 20\text{ mins})\)
Nucleosynthesis is the stage where light elements (i.e., Hydrogen, Deuterium, Helium, Lithium) were formed. Elements heavier than Helium were very difficult to form due to the large amounts of energy/time required.
- Matter domination \((t = 47,000\text{ years})\)
Around 47,000 years after the Big Bang, the energy density of matter in the Universe overtakes that of radiation. Matter now becomes the dominant driver of the Universe’s evolution.
- Epoch of recombination and photon decoupling \((t = 377,000\text{ years})\)
During the epoch of recombination, ionised particles (e.g. electrons, protons) combined to form neutral atoms. At this stage, the matter density was such that photons only had a short path length before they would interact with an ionised particle, making the Universe opaque. As recombination progressed, and ionised particles combined into neutral atoms, the photon path length increased, making the Universe transparent to photons. The decoupled photons produced during this time have been redshifted by the expansion of the Universe, so we now observe them as the Cosmic Microwave Background (CMB).
- Dark Ages \((380,000 \text{ years}< t < 1\text{ Gyr})\)
At this time, photons were free to stream through the Universe. However, light-producing systems such as stars had not yet formed, so the Universe was "dark". The only sources of photons were those from the CMB, and those emitted from neutral Hydrogen atoms at 21 cm.
- Formation of structure \((150\text{ Myr} < t < 1\text{ Gyr})\)
Structure forms hierarchically in the Universe; smaller structures form first and are built up into larger ones. The first structures to form in the Universe were stars, dwarf galaxies, and quasars. The first population of stars (Population III stars) are thought to have formed around t \(=700\) Myr. Pop III stars are yet to be detected observationally.
- Epoch of reionisation \((250 \text{ Myr} < t 1\text{ Gyr})\)
Pop III stars introduced a new source of radiation into the Universe. During the epoch of reionisation this radiation ionised the neutral Hydrogen, creating a plasma of protons and electrons. The epoch of reionisation most likely lasted until around 1 Gyr, when the Population III stars died off. At this time, the ionised Hydrogen gradually recombined to neutral Hydrogen once more.
- Formation of Galaxies and Clusters \((t\)>\(1 \text{ Gyr})\)
"Large" galaxies and galaxy clusters formed from around t = 1 Gyr. These would have first comprised Population II stars, which, unlike Pop III stars, we do observe today. Later, the next generation of stars, Pop I, formed.
- Dark Energy epoch \((t > 9.8 \text{ Gyr})\)
We are currently believed to be in the dark energy epoch, where the energy density of the Universe is dominated by the dark energy contribution. While we don’t know the nature of dark energy, its effects are seen as driving the expansion of the Universe so it is not just getting larger, but the expansion itself is accelerating.
1.5 The Expanding Universe
The first observational evidence that the Universe is expanding was found by Edwin Hubble (Hubble 1929). By measuring the distances to nearby galaxies using the Cepheid Leavitt Law, and comparing these distances to the galaxies’ redshifts. Hubble measured the expansion rate of the Universe (the Hubble constant, \(\mathbf{H_0}\)) to be approximately 500 km s\(^{-1}\) Mpc\(^{-1}\). Hubble’s observations are shown in Figure 1.3. Throughout this course we will explore how observations of the Universe have developed over the past century, increasing in precision and accuracy, leading to our current understanding of Cosmology.
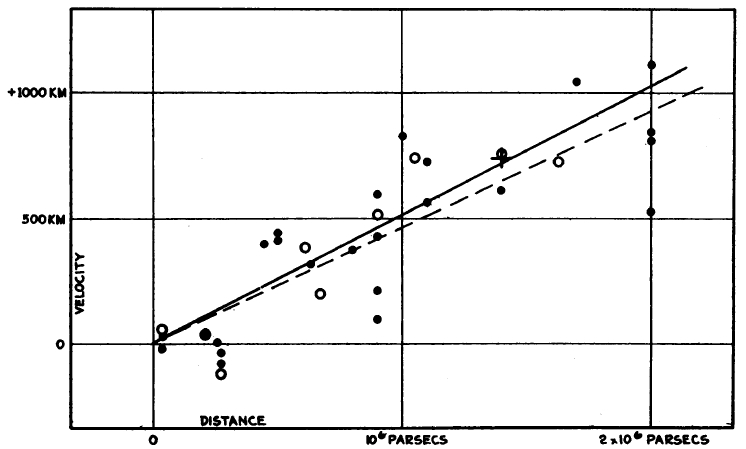
Figure 1.3: The first observational evidence of the expanding Universe. His first measurement of the expansion rate, now known as the Hubble constant, was 500 km s\(^{-1}\) Mpc\(^{-1}\). From Hubble (1929).
References
Hubble, Edwin. 1929. “A Relation between Distance and Radial Velocity among Extra-Galactic Nebulae.” Proceedings of the National Academy of Science 15: 168.